I.4 Amino Acid Analysis
The first step in analyzing a polypeptide is hydrolysis and
quantitative determination of its amino acid composition. Recall that
amide bonds are very resistant to hydrolysis. Hydrolysis of
polypeptides requires heating in 6M HCl at 110°C for 24-70 hr or
heating 2-4M NaOH at comparable temperature and times. Once the
polypeptide is hydrolyzed, the resulting mixture of amino acids is
analyzed by ion-exchange chromatography. Amino acids are detected as
they emerge from the column by reaction with ninhydrin (See previous).
Current procedures for hydrolysis of polypeptides and analysis of amino
acid mixtures have been refined to the point where it is possible to
obtain amino acid composition from as little as 50 nanomoles
(50•10—9 mol) of polypeptide. Figure I.5 shows the analysis of a
polypeptide hydrolysate by ion exchange chromatography. Note that
during hydrolysis, the side-chain amides of asparagine and glutamine
are hydrolyzed and these amino acids are detected as glutamic acid and
aspartic acid. For each glutamine or asparagine hydrolyzed, there is an
equivalent amount of ammonia formed.
Absorbance
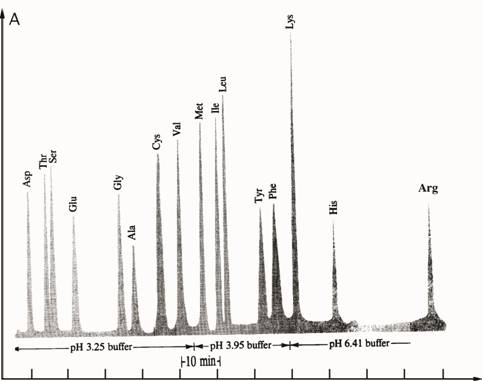
Time
Figure I.5 Analysis of mixture of amino acids by
ion-exchange chromatography.
I.5 Primary Structure of Polypeptides and Proteins
Primary (1°) structure of polypeptides and proteins
refers to the sequence of amino acids in a polypeptide chain. In this
sense, primary structure is a complete description of all covalent
bonding in a polypeptide or protein.
It is difficult to appreciate the incredibly large number of different
polypeptides that can be constructed from 20 amino acids, where the
number of amino acids in a polypeptide can range from under ten to well
over a hundred. With only three amino acids, there are 27
different tripeptides possible. For glycine, alanine
and serine, the 27 tripeptides are:
gly—gly—gly
ser—ser—ser ala—ala—ala
gly—gly—ser
ser—ser—gly ala—ala—gly
gly—gly—ala
ser—ser—ala ala—ala—ser
gly—ser—gly
ser—gly—ser ala—gly—ala
gly—ala—gly
ser—ala—ser ala—ser—ala
gly—ser—ala
ser—gly—ala ala—gly—ser
gly—ala—ser
ser—ala—gly ala—ser—gly
gly—ser—ser
ser—gly—gly ala—gly—gly
gly—ala—ala
ser—ala—ala ala—ser—ser
2
Statistical analyze of human protein average polypeptide chain
length shows number Npeptide = 184 of the
20 = nAA different amino acids
(Combinations-Variations) Comb, the number of
possible polypeptides is
; m=Npeptide-i*nAA= 4
truly countless variation-combination of
20 amino acids arrangement on the polypeptide chain of 184 AA starting
from N-terminal and finishing on C-terminal.
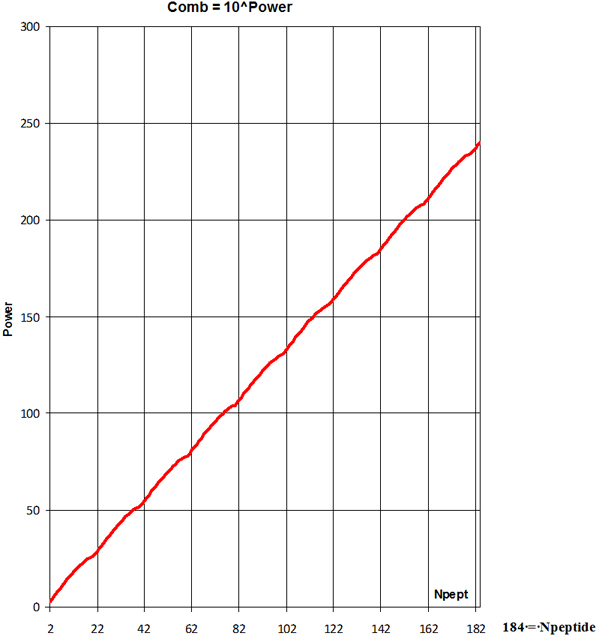
3
I.6 Three-Dimensional Shapes of polypeptides and Proteins A.
Geometry of a Peptide Bond
In the late
1930s, Linus Pauling began a series of studies designed to learn more
about the three-dimensional shapes of proteins. One of his first
discoveries was that a peptide bond itself is planar. As shown in
Figure I.6, the four atoms of a peptide bond —CO—HN— and
the two alpha-carbons joined to it all lie in the same plane —Cα—CO—HN—Cα—.
Had you been asked earlier to predict the geometry
of a peptide bond, you probably would have reasoned in the
following way. There are three bundles of electron density around the
carbonyl carbon; therefore predict bond angles of 120° about the
carbonyl carbon. There are four bundles of electron density around the
amide nitrogen; therefore predict bond angles of 109.5° about this
atom. These predictions agree with the observed bond angles of
approximately 120° about the carbonyl carbon. However, a bond angle of
120° about the amide nitrogen is unexpected. To account for this
observed geometry, Pauling proposed that a peptide bond is more
accurately represented as a resonance hybrid of two important
contributing structures:
I
II
Figure I.6 Planarity of a peptide bond. Bond angles
about the carbonyl carbon and the amide nitrogen are
approximately 120°.
Contributing structure I show C=O
double bond and a C—N
single bond. Structure II shows a C—O
single bond and a C=N
double bond. If structure I is the major contributor to the hybrid, the
C—N—C bond angle would
be nearer 109.5°. If, on the other hand, structure II is the major
contributor, the C—N—C
bond angle would be nearer 120°. The fact, first observed by Pauling,
is that the C—N—C bond
angle is very near 120°, which means that the peptide bond is planar
and structure II is the major contributor to the resonance hybrid.
Two configurations are possible for the atoms of a planar peptide bond.
In one configuration, the two
a-carbons are cis to each other; in the other, they
are trans to each other:
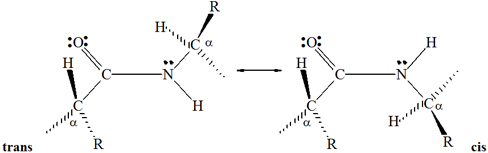
The trans configuration is more favorable because
the bulky a-carbons are farther from each other than they are in the cis
configuration. Virtually all peptide bonds in natural proteins have the
trans configuration.
4
B. Secondary Structure
Secondary (2°) structure refers to ordered
arrangements (conformation) of amino acids in localized regions of a
polypeptide or protein, molecule. The first studies of polypeptide
conformations were also carried out by Linus Pauling and Robert Corey,
beginning in 1939. They assumed that in conformations of greatest
stability,
(1) all atoms in a peptide bond lie in the same
plane and (2) each amide group is
hydrogen-bonded between the
N—H of
one peptide bond and the C=O
of another, as shown in Figure I.7. On this basis of model-building,
Pauling and Corey proposed that two folding patterns should be
particularly stable: the α-helix and the antiparallel
β-pleated sheet. In the a-helix pattern shown
in Figure I.7, a polypeptide chain is coiled in a spiral.
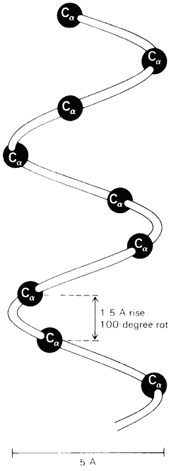
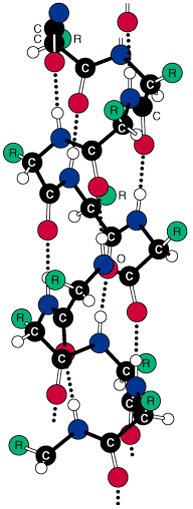
Figure I.7 (a) A
right-handed α-helix space-filling
α-C model on the carbon-nitrogen backbone trace
of an α-helix. (b)
Ball-and-stick model of an α-helix
showing intra chain hydrogen bonds •••.
a-helix in one turn has 3.6 amino
acid residues and one step amino acid turn stage have 1.5 Å and 100 º
angle.
As you study the a-helix in Figure I.7, note the following:
1. The helix is coiled in a clockwise or right-handed
manner. Right-handed means that if you turn the helix clockwise, it
twists away from you. In this sense, a right-handed helix
is analogous to the right-hand thread of a common wood or machine screw.
2. There are 3.6 amino acids per turn of the helix.
3. Each peptide bond is trans and planar.
4. The N—H
group of each peptide bond points roughly upward, parallel to the axis
of the helix; and the C=O
of each peptide bond points roughly downward, also parallel to the axis
of helix.
5. The carbonyl group of each peptide bond is
hydrogen-bonded to the N—H
group of the peptide bond four amino acid units away from it. Hydrogen
bonds are shown as dotted lines.
6. All R— groups point outward from
the helix.
Almost immediately after Pauling proposed the α-helix
structure, other researchers proved the presence of
α-helix in keratin, the protein of
hair and wool. It soon became obvious that the α-helix
is one of the fundamental folding patterns for secondary
structure 2º of polypeptide chains.
5
β-pleated sheets consist of
extended polypeptide chains with neighboring chains running in opposite
(antiparallel) directions. Unlike the a-helix
arrangement, N—H and C=O groups lie in the sheet and are
roughly perpendicular to the long axis of the sheet. The C=O group of each peptide bond is
hydrogen-bonded to the N—H group of a peptide
bond of a neighboring chain C=O- -
-H—N.
Amino terminus —————> Polypeptide chain ——————> Carboxyl terminus
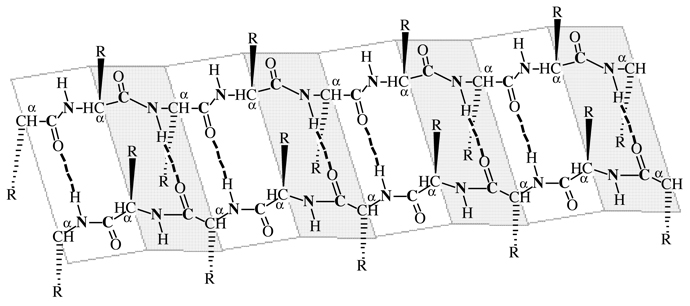
Carboxyl terminus <—————— Polypeptide chain <———————— Amino terminus
Figure I.8 β-pleated
sheet conformation with two polypeptide chains running in
opposite (antiparallel)
directions. Hydrogen bonding between chains is indicated by dotted
lines C=O- - -H—N.
As you study the secondary 2º structure of β-pleated
sheet shown in figure I.8, note the following:
1. The two polypeptide chains lie adjacent to each
other and run in opposite (antiparallel) directions.
2. Each peptide bond is trans and planar.
3. The polypeptide is a chain of flat or planar
sections connected at amino acid α-carbons.
4. The C=O
and N—H groups of
peptide bonds from adjacent chains point at each other and are in the
same plane, so hydrogen bonding is possible between adjacent
polypeptide chains.
5. The R— groups on any one chain
alternate, first above the plane of the sheet and below the plane of
the sheet.
The pleated sheet conformation is stabilized by
hydrogen bonding between N—H
groups of one chain and C=O
groups of an adjacent chainC=O- -
-H—N. By comparison, the α-helix
is stabilized by hydrogen bonding between
N—H and C=O groups within the same polypeptide
chain.
Secondary 2º structure is used to describeα-helix,
b-pleated sheet and other types of
periodic conformations in localized regions of polypeptide or protein
molecules like as beta turns or loops
as well bents.
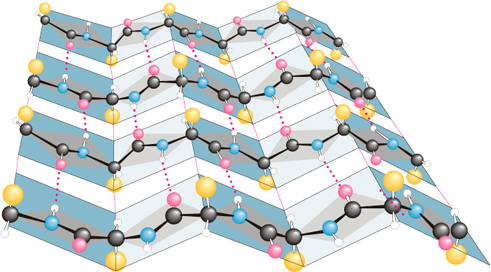
6
Loops, Turns & Bends
Roughly half of the residues in a "typical" globular protein reside
in α-helices and β-sheets but
second half in loops, turns, bends
and other extended conformational features. Loops, turns
and bends refer to short segments of amino acids that
join two units of secondary structure, such as two adjacent strands of
an antiparallel β-sheet. A
β-turn
involves four aminoacyl residues, in which the first residue is
hydrogen-bonded to the fourth, resulting in a tight 180-degree turn
(Figure 5–7). Proline and glycine often are present in β-turns.
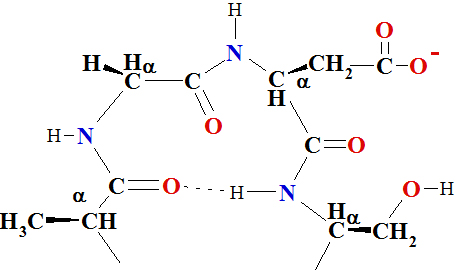
The term secondary structure is used to describe α-helix,
β-pleated sheet and β-turns of
periodic conformations in localized regions of polypeptide or protein
molecules.
C. Tertiary Structure
Tertiary (3°) structure refers to the overall
folding pattern and arrangement in space of all atoms in a single
polypeptide chain. Actually, there is no sharp dividing line between
secondary and tertiary structure. Secondary structure refers to the
spatial arrangement of amino acids close to one another on a
polypeptide chain and tertiary structure refers to the
three-dimensional arrangement of all atoms of a polypeptide chain.
Disulfide bonds (Section of Thiols) are important in
maintaining tertiary structure. Disulfide bonds are
formed between side chains of cysteine by oxidation of two thiol groups
(Cys—SH)
to form
the disulfide bond (Cys—S—S—Cys)
, as shown for cysteine containing polypeptide parts:

cysteine units on adjacent polypeptide
chains disulfide
bond (bridge) between cysteine thiol groups
Treatment of a disulfide bond with a reducing
agent regenerates the thiol group that is reduction.
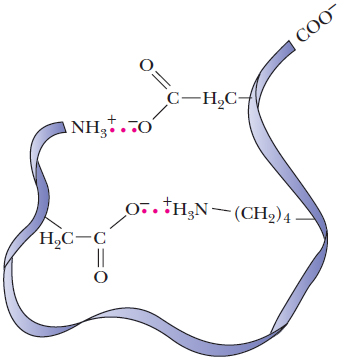
7
The four important structural features of myoglobin
are:
1. The backbone consists of eight
relatively straight sections of a-helix A, B,
C, D, E, F, G, H, each separated by a β-bend
in the polypeptide chain. The longest section of α-helix
has 23 amino acids, the shortest has 7. Some 75% of the amino acids are
found in these eight regions of α-helix.
2. Hydrophobic side chains, such as those of
phenylalanine, alanine, valine, leucine, isoleucine and methionine are
clustered in the interior of the molecule, which shield oxygen O=O from contact with
water and hydroxonium ions
. Hydrophobic interactions
between nonpolar side chains are important in directing the folding of
the polypeptide chain of myoglobin into this compact,
three-dimensional shape.
3. The outer surface of myoglobin is
coated with hydrophilic side chains, such as those of lysine, arginine,
serine, glutamic acid, histidine and glutamine, which interact with the
aqueous environment create water soluble hydrate coat.
The only polar side chains that point to the myoglobin
molecule are those of two histidines. These side chains can be seen in
Figure I.9 as five-member single rings pointing inward toward the heme
group.
4. Oppositely charged amino acids close to each other
in the three-dimensional structure interact by electrostatic
attractions called salt linkages or salt
bridges. An example of a salt bridge is the
attraction of the side chains of lysine amino
and
glutamic acid carboxylic
groups.
The tertiary structures of several other globular proteins have
also been determined. It is clear that globular proteins
contain a-helix and β-pleated
sheet structures and also that the relatively amounts of
each vary widely. Lysozyme, with 129 amino acids in a
single polypeptide chain, has only 25% of its amino acids in α-helix
regions. Cytochrome, with 104 amino acids in a single
polypeptide chain, has no α-helix structure but does
contain several regions of
β-pleated sheet. Yet, whatever the
proportions of α-helix, β-pleated
sheet or other periodic structure, virtually all nonpolar side
chains of globular proteins are directed toward the interior of the
molecule, while polar side chains are on the surface of the molecule
and are in contact with the aqueous environment. Thus the same type of hydrophobic/hydrophilic
interaction that are responsible for formation of soap micelles
(See Surface active compounds) and phospholipid bilayers (Will see on
next lecture) are responsible for the three-dimensional shapes of globular
proteins.
Example I.5 With which of the following amino acid
side chain can the side chain of threonine form hydrogen bonds?
a. valine b. asparagine c.
phenylalanine d. histidine e.
tyrosine f. alanine . Solution The side
chain of threonine contains a hydroxyl group —OH
that can participate in hydrogen bonding in two ways:
oxygen has a partial negative charge and can function as a hydrogen
bond acceptor; hydrogen has a partial positive charge and can function
as a hydrogen bond donor. Therefore, the side chain
of threonine can function as a hydrogen bond acceptor
for the side chain of tyrosine, asparagine and histidine. The side
chain of threonine can also function as a hydrogen bond
donor for the side chains of tyrosine, asparagine and histidine.
Problem I.5
At pH=7.4, with amino acid side chains can the side chain of lysine
form salt linkages?
8
D. Quaternary 4º Structure
Most proteins of molecular weight greater than 50 000 consist of two
or more with five 5 intermolecular forces linked
polypeptide chains. The arrangement of protein monomers in an
aggregation is known as quaternary (4°) structure. A
good example is hemoglobin, a protein that consist of four separate
protein monomers: two α-chains of 141 amino acids
each and two b-chains of 146 amino
acids each. The quaternary (4°) structure of
hemoglobin is shown in Figure I.11.
beta chains
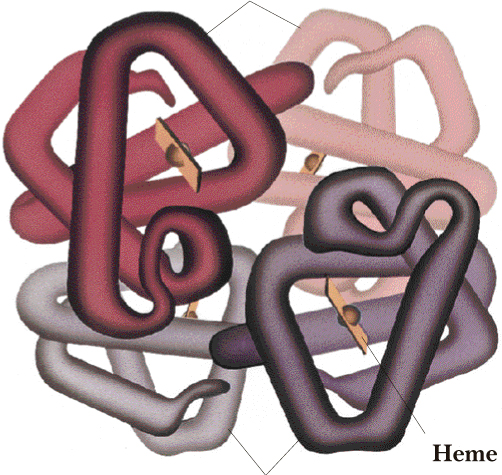
alpha chains
Figure I.11 The quaternary (4°) structure
of hemoglobin, showing the four subunits packed together. The flat disk
represent four heme units.
The chief factor stabilizing the aggregation of protein subunits is hydrophobic
interaction. When separate monomers fold into compact
three-dimensional shapes to expose polar side chains to the aqueous
environment and shield non-polar side chains from water, there are
still hydrophobic "patches" on the surface,
in contact with water. These patches can be shield from water if two or
more monomers assemble so their hydrophobic patches
are in contact. The molecular weights, numbers of subunits and
biological functions of several proteins with quaternary (4°)
structure are shown in Table I.7
Table I.7
Quaternary
|
Protein
|
Mol.Wt.
|
Number
of Subunits
|
Subunit
Mol.Wt.
|
Biological Function
|
structure
of selected
proteins.
|
insulin
hemoglobin
alcohol dehydrogenase
lactate dehydrogenase
aldolase
fumarase
tobacco mosaic virus
|
11 466
64 500
80 000
134 000
150 000
194 000
40 000 000
|
2
4
4
4
4
4
2280
|
5 733
16 100
20 000
33 500
37 500
48 500
17 500
|
a hormone regulating
glucose metabolism
oxygen transport in blood plasma
an enzyme of alcoholic
fermentation
an enzyme of anaerobic glycolysis
an enzyme of anaerobic glycolysis
an enzyme of the tricarboxylic
acid
Krebs cycle
plant virus coat
|
9
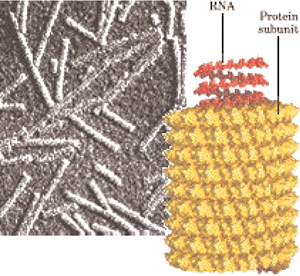
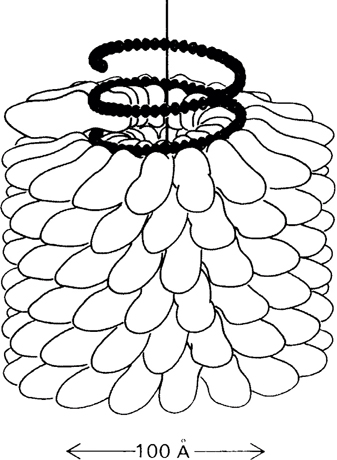
Figure I.12a The quaternary
structure of tobacco mosaic virus
built up from 2200 protein subunits and
wrapping up the informative chain of ribonucleic acids RNA,
particularly built in quaternary helical symmetry structure of proteins
like to hollow cylinder 3000
long size and 180
external
diameter size.
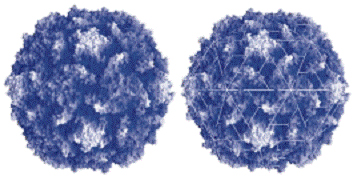
Figure I.12b The quaternary structure of polio
virus capsids built up from protein subunits stuck in
icosahedral rotational symmetry by 300
size and wrapping up the
informative chain of ribonucleic acids RNA,
particularly built in quaternary structure of proteins.
10
E. 1° Structure Determines 2°, 3° and 4° Structure
The primary structure of a protein is determined by information
coded within genes. Once the primary structure of a polypeptide is
established, the structure itself directs the folding of the
polypeptide chain into a three-dimensional structure. In the other
words, information inherent in the primary structure of a protein
determines its secondary, tertiary and quaternary structures.
If three-dimensional shape of a polypeptide or protein is determined by
its primary structure, how can we account for the observation that denaturation
is irreversible for some proteins and not others?
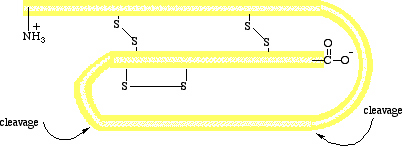
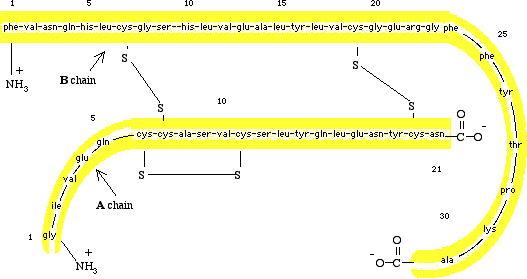
Figure I.13 (Top) A schematic diagram of pro
insulin, a single polypeptide chain of 84 amino acids.
(Bottom) The amino acid sequence of bovine insulin.
The reason for this difference in behavior from one protein to
another is that some proteins, like ribonuclease, are synthesized as
single polypeptide chains, which then fold into unique
three-dimensional structures with full biological activity. Others,
like insulin, are synthesized as larger molecules that are not
biologically active at first but "activated" later by specific
enzyme-catalyzed peptide-bond hydrolize. Insulin is synthesized in the
beta cells of the pancreas as a single polypeptide chain of 84 amino
acids. This molecule, called pro insulin, has no biological activity.
When insulin is needed, a section of 33 amino acids is hydrolyzed from
pro insulin in an enzyme-catalyzed reaction to produce the active
hormone (Figure I.13). Bovine insulin contains 51 amino acids in two
polypeptide chains. The A chain contains 21 amino
acids and has glycine (Gly[G])at the
terminus
and asparagine (Asn[N]) at the
terminus. The B chain contains 30
amino acids with phenylalanine (Phe[F])
at the
terminus
and alanine (Ala[A]) at the
terminus.
11
Because the information directing the original folding of the single
polypeptide chain of pro insulin is not present in the A
and B chains of the active hormone,
refolding of the denatured protein is irregular and denaturation is
irreversible.
Zymogens are enzyme produced as inactive proteins, which are
then activated by hydrolize of one or more of the polypeptide bonds.
The process of producing a protein in an inactive, storage form is
common. For example, the digestive enzymes trypsin and chymotrypsin are
produced in the pancreas as inactive proteins, named trypsinogen and
chymotrypsinogen. There is a logical and simple reason for the
synthesis of zymogens. In the case of trypsin and chymotrypsin, their
function is to catalyze the hydrolysis of dietary proteins reaching the
intestinal track. Proteins there are hydrolyzed to their component
amino acids and then absorbed through the wall of the intestine into
bloodstream. If trypsin and chymotrypsin were produced as active
enzyme, they might well catalyze their own hydrolysis as well as that
of other proteins in the pancreas-in effect, a "self-destruct" system.
But nature has protected against this happening by synthesizing and
storing zymogens instead.
F. Denaturation
Globular proteins found in living organisms are remarkably sensitive
to changes in environment (pH, T, SAC, solvent composition, mechanical
treatment, urea). Relatively small changes in pH, temperature or
solvent composition, even for only a short period, may causes them to
become denatured. Denaturation
causes mechanical-physical activity. Except for cleavage of disulfide
and coordinative bonds, denaturation
stems from changes in secondary, tertiary
or quaternary structure through disruption of non
covalent intermolecular interaction forces, such as hydrogen
bonds, salt linkages and hydrophobic
bonds. Common denaturing agents include the
following:
1. Heat. Most globular proteins become denatured
when heated above T > 50-60° C. For example, boiling or frying an
egg causes egg-white and yolk proteins to become denatured,
forming an insoluble mass.
2. Large change in pH. Adding concentrated
acid or alkali to a protein in aqueous solution causes changes in the
charged character of ionizable side chains and interferes with salt
linkages. For example, in certain clinical chemistry tests
where it is necessary first to remove any protein material,
trichloroacetic acid (a strong organic acid) is added to denature
and precipitate any protein present.
3. Detergents. Treating a protein with surface
active compounds (SAC) like as sodium dodecylsulfate (SDS),
(See next lecture), a detergent, causes the native conformation
to unfold and exposes the nonpolar protein side chains to the aqueous
environment. These side chains are then stabilized by hydrophobic
interaction with hydrocarbon chains of the detergent.
4. Organic solvents such as alcohols,
acetone or ether.
5. Mechanical treatment. Most globular
proteins are denatured in aqueous solution if they
are stirred or shaken vigorously. An example is whipped egg whites to
cook beze biscuits or pudding.
6. Urea and guanidine hydrochloride cause
disruption of protein hydrogen bonding and hydrophobic
interactions. Because urea is a small molecule with a high
degree of polarity, it is very soluble in water. A solution of 8M urea
(480 g urea/L of water) is commonly used to denature
proteins. Guanidine is a derivative of urea in which >C=O is replaced by =NH. Guanidine is a strong
base and reacts with HCl
and to form the salt guanidine hydrochloride.
Denaturation can be partial or complete. It can
also be reversible or irreversible. For example, the hormone insulin
can be denatured with 8M urea and then the
three disulfide bonds Cys—S—S—Cys
reduced to six free Cys—SH
groups. If urea is then removed and the disulfide bonds
re-formed by oxidation, the resulting molecule has less than 1% of its
former biological activity. In this case, denaturation
is both complete and irreversible. Consider another example,
ribonuclease, an enzyme that consist of a single polypeptide chain of
124 amino acids folded into a compact, three-dimensional structure
partly stabilized by four disulfide bonds Cys—S—S—Cys.
Treatment of ribonuclease with urea causes the molecule to
unfold, and the disulfide bonds can then be reduced
to thiol groups Cys—SH.
At this point, the protein is completely denatured-it
has no biological activity. If urea is removed from solution
and the thiol groups Cys—SH
reoxidized to disulfide bonds Cys—S—S—Cys,
the protein regains its full biological activity. In this instance, denaturation
has been complete but reversible.
12
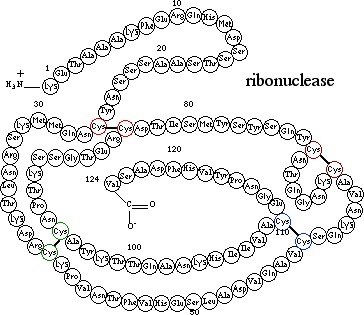
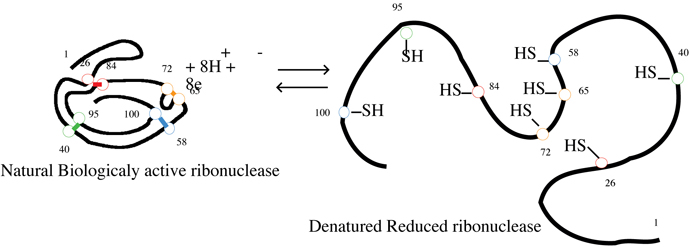
I.7 Fibrous Proteins
Fibrous proteins are stringy, physically tough
macromolecules composed of rod like polypeptide chains joined together
by several types of cross-linkages to form stable, insoluble
structures. The two main classes of fibrous proteins
are the keratins of skin, wool, claws, horn and feathers and the
collagens of the tendon and hides.
A. The Alpha-Keratins
Hair and wool are very flexible and also elastic, so when tension is
released, the fibers revert to their original length.
At the molecular level, the fundamental structural unit of hair is a
polypeptide wound into an α-helix
conformation (Figure I.14). Several levels of structural organization
are built from simple α-helix. First,
three strands of α-helix are twisted
together to form a larger cable called a protofibril. Protofibrils
are then wound into bundles to form an 11-strand cable called a micro
fibril. These, in turn, are embedded in a larger matrix that
ultimately forms a hair fiber (Figure I.14). When
hair is stretched, hydrogen bonds along turns of each
a-helix are elongated. The main force
causing stretched hair fibers to return to their
original length is re-formation of hydrogen bonds in
the α-helice.
The a-keratins of horns and claws
have essentially the same structure as hair but with a much higher
concentration of cysteine (Cys) and greater degree of disulfide
cross-linking Cys—S—S—Cys
between individual helices. These additional disulfide
bonds Cys—S—S—Cys
greatly increase resistance to stretching and produce the hard keratins
of horn and claw.
13
B. Collagen Triple Helix
Collagens are constituents of skin, bone, teeth,
blood vessels, tendons, cartilage and connective tissue. They are the
most abundant protein in higher vertebrates and make up almost 30% of
total body mass in humans.
Table I.8 lists the collagen content of several
tissues. Note that bone, the Achilles tendon, skin, and the cornea of
the eye are largely collagen.
Table I.8
Collagen content |
Tissue
|
Collagen
(% Dry Weight)
|
Tissue
|
Collagen
(% Dry Weight)
|
of some body
tissues
|
bone, mineral-free
Achilles tendon
skin
cornea
|
88
86
72
68
|
cartilage
ligament
aorta
|
46-63
17
12-24
|
Because collagen is abundant and widely
distributed in vertebrates and because it is associated with a variety
of diseases and problems of aging, more is known about this fibrous
protein than about any other. Collagen molecules are
very large and have a distinctive amino acid composition. One-third of
all amino acids in collagen are glycine and
another 21% are either hydroxylysine or hydroxyproline (See former
amino acids). Both hydroxylated amino acids are formed after their
parent amino acids (L-proline and L-lysine) and incorporated in collagen
molecules. Because cysteine is almost entirely absent, there are no disulfide
cross-links in collagen. When collagen
fibers are boiled in water, they are converted to
insoluble gelatins.
The polypeptide chains of collagen fold into a
conformation that is particularly stable and unique to collagen.
In this conformation, three protein strands wrap around each other to
form a left-handed super helix called the collagen
triple helix. This unit, called tropocollagen,
looks much like a three-stranded rope (Figure I.15).
The hydroxyl groups of hydroxyproline and hydroxylysine residues help
to maintain the triple helix structure by forming hydrogen
bonds >C=O•••H—N< between adjacent chains. Fibers
in which proline and lysine groups have not been hydroxylated are far
less stable than fibers in which these groups have
been hydroxylated. one of the important functions of vitamin C is in
hydroxylation of collagen. Without adequate supplies
of vitamin C, collagen metabolism is impaired, giving
rise to scurvy, a condition in which tropocollagen fibers
do not form stable physically tough fibers. Scurvy
produces skin lesions, fragile blood vessels and bleeding gums.
Collagen fibers are formed when many
tropocollagen molecules line up side by side in
regular pattern and are then cross-linked by newly
formed covalent cross-bonds. Most covalent cross-linking
involves the side chains of lysines. The extent and type of cross-linking
vary with age and physiological condition. For example, the collagen
of rat Achilles tendon is highly cross-linked and collagen
of the more flexible tendon of rat tail is less highly cross-linked.
Further, it is not clear when, if ever, the process of cross-linking
is completed. Some believe it continues throughout life, producing
increasingly stiffer skin, blood vessels and other tissues, which then
contribute to the medical problems of aging and the aged, making more
fragile bones and tissues.
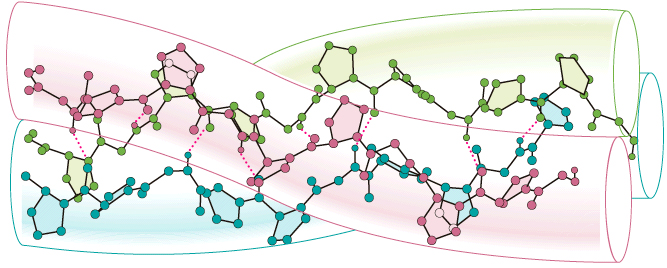
I.8 Plasma Proteins: Examples of Globular Proteins
Human blood consists of a fluid water solution portion (plasma) and
cellular components. The cellular components, which make up 40-50% of
volume of whole blood, consist of red blood cells (erythrocytes), white
blood cells (leukocytes) and blood platelets (trombocytes). Human
plasma consist largely of water (90-92%) in which are dissolved various
inorganic ions and a heterogeneous mixture of organic molecules, the
largest groups of which are the plasma proteins. The
earliest method of separating plasma proteins into
fractions used ammonium sulfate to "salt out" different types of proteins.
The fraction precipitated from plasma 50% saturated with ammonium
sulfate was called globulin. The fraction not
precipitated at this salt concentration but precipitated from plasma
saturated with ammonium sulfate was called albumin.
Today, electrophoresis is the most common method of
separating proteins of biological fluids into
fractions, especially in the clinical laboratory, where it is used
routinely to measure proteins in human plasma, urine
and cerebrospinal fluid. It is estimated that between 15 and 20 million
plasma-protein electrophoretic
analyses are carried out each year in the United States and Canada.
In plasma-protein electrophoresis, a sample of plasma
is applied as a narrow line to a cellulose acetate strip. The ends of
the strip are then immersed in a buffer of pH 8.8 and a voltage is
applied to the strip. At pH 8.8, plasma proteins have
net negative charges and migrate toward the positive electrode. After a
predetermined time, the cellulose acetate strip is removed, dried and
sprayed with a dye that selectively stains proteins.
The separated protein fractions then appear on the
developed strip as spots (Figure I.16 (a) ). The amount of protein
in each spot is determined using a densitometer to measure intensity
versus width of each peak.
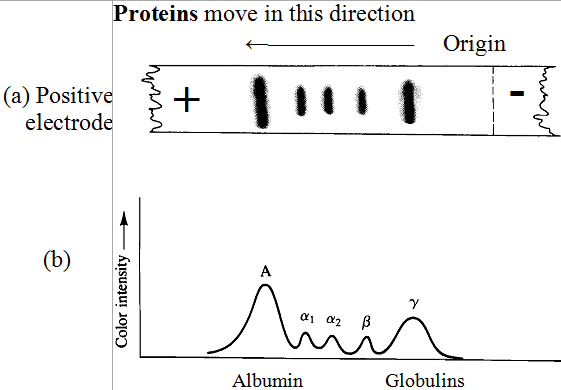
Electrophoresis on cellulose acetate separates
serum proteins into five large fractions: one albumin
fraction and four globulin fractions. The four globulin
fractions are arbitrarily designated α1,
α2, β and γ
according to their electrophoretic mobilities. Serum albumin
has an isoelectric point of about pH 4.9 and migrates
farthest toward the positive electrode. Gamma-globulin
has an isoelectric point of about pH 7.36 and
migrates the shortest distance. Shown in table I.9 are the
concentrations of the five large protein fractions of
human serum.
The primary function of albumins is to regulate
the osmotic pressure of blood. In addition, albumins
are important fatty acids and certain drugs such as aspirin and
digitalis. The α1 and α2 fractions
transport other biomolecules, such as fats, steroids and phospholipids
and various other lipids.
Table I.9
Concentrations of
|
Fraction
|
(g/100 mL)
|
Total
Protein (%)
|
the important
human serum
proteins as
determined by
electrophoresis.
|
albumin
globulins
α1
α2
β
γ
|
3.5-5.0
0.1-0.4
0.5-1.1
0.6-1.2
0.5-1.5
|
52-67
2.5-4.5
6.6-13.6
9.1-14.7
9.0-21.6
|
The α1 fraction also contain
antitrypsin, a protein that inhibits the protein-digesting enzyme
trypsin. The α2 fraction
contains haptoglobulin, which binds any hemoglobin
released from destroyed red blood cells and ceruloplasmin,
the principal copper-containing protein of the body.
The α2 fraction also contains prothrombin,
an inactive form of the blood-clotting enzyme thrombin. The β
fraction contains a variety of specific transport proteins,
as well as substances involved in blood clotting.
The γ-globulin
fraction consists primarily of antibodies (immunoglobulins),
whose function is to combat antigens (foreign or
undesirable proteins for organism) introduced into
the host body. Specific antibodies are formed by the
immune system in response to specific antigens. The
response is the basis for immunization against such infectious diseases
as polio, tetanus and diphtheria. An antibody consist
of a combination of two heavy (high-molecular-weight)
and two light (low-molecular-weight) polypeptide
chains held together by four disulfide bonds Cys—S—S—Cys (Figure I.17). Each antibody
has two identical binding sites paratops that react
with specific antigens to form an insoluble complex
called precipitin. Formation of precipitin
deactivates the antigen and permits its removal and
breakdown by white blood cells (leucocytes-macrophages).
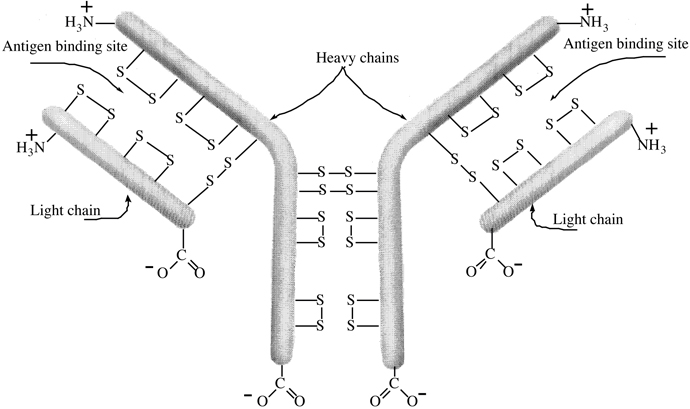
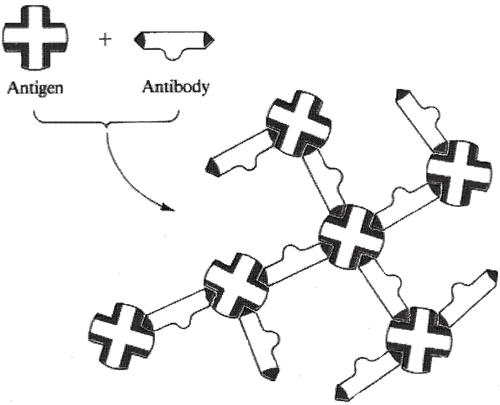
Precipitin complex
(insoluble)
Figure I.17 The projection of three-dimensional shape antibody.
The iteraction between antibody and its specific antigen
to form an inactive precipitin
complex. The precipitated antigen-antibody complex is
then ingested and broken down whit blood cells.